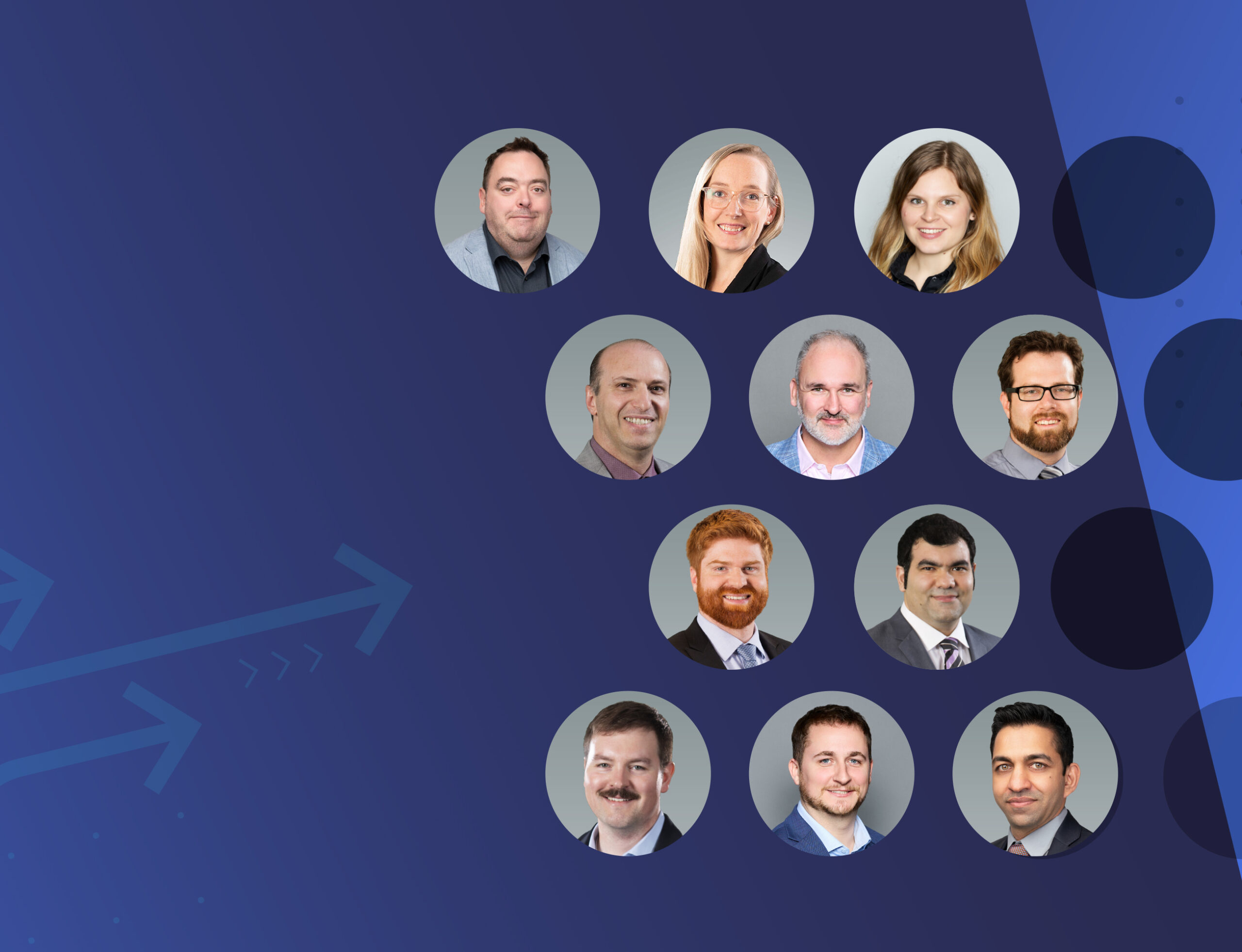
HH Angus is pleased to announce the appointment of several new Principals and Associates to the firm’s leadership, and to recognize their contributions to our growth and business practices. Their demonstrated excellence in client service, continuous professional development, and mentorship of their team members strongly support our values and goals.
“The appointment of these deserving individuals reflects their commitment to advancing our technical expertise, innovation, outstanding project delivery, development of strong client and industry relationships, and motivating their colleagues to excel”, comments Paul Keenan, President, HH Angus.
We congratulate all our new Principals, Senior Associates and Associates for achieving these exceptional career milestones, and for their contributions to the growth of the firm’s leadership, as well as to the ongoing expansion of our services and portfolio across Canada and internationally.
OUR NEW PRINCIPALS
Andy Crosson, Associate Director – Angus Connect
Andy quickly assumed a leadership role when he joined HH Angus and currently carries dual responsibilities for market development and management of major pursuits and projects throughout Western Canada and across the country. With 25+ years of industry experience, he has acquired an excellent grasp of both the opportunities digital technologies offer to building owners, and the practical implications of implementing these in design and construction – from new construction to renovations/retrofits of existing buildings. From projects in North America, the UK and the Middle East, Andy brings deep knowledge of and expertise in design, project management and delivery of major complex projects, specializing in telecommunications, audio visual, smart buildings and network design. Some of Andy’s notable projects include New Surrey Hospital and BC Cancer Centre, Burnaby Hospital Phase 2, operational readiness consulting for Dawson Creek and District Hospital Replacement, UHN’s Toronto Western Hospital New Surgical and Patient Tower, and Burrard Exchange in Vancouver for Hudson Pacific Properties.
Andy:
“I am excited and grateful to join the ownership group at HH Angus and am looking forward to continue working with our growing team in BC to deliver high quality, meaningful and impactful projects with our clients and partners.”
Chris Piché, Vice President – Commercial Division
Chris brings over 24 years of leadership in high-performance design and the delivery of energy-efficient, low-carbon solutions. A former member of the Board of Directors for the Canada Green Building Council, Chris is deeply committed to environmentally responsible design that evolves with advancing technology and aligns with client goals and project objectives. Recognized for strength in stakeholder engagement, Chris excels at building strong relationships and ensuring alignment between project goals, client expectations, and regulatory requirements—while balancing technical, financial, and sustainability priorities. He leads HH Angus’ growing Commercial Division, which delivers a wide range of services including base building engineering, tenant improvements, vertical transportation, lighting design, ICAT/IMIT, sustainability consulting, energy modeling, commissioning, fire protection, smart buildings, and more—across sectors from office towers and post-secondary campuses to airport terminals and sports venues.
Chris:
“I'm grateful for the opportunity to step into this role and for the trust and support that made it possible. HH Angus is the ideal place to continue championing people and community-focused design—creating spaces that serve, inspire, and drive lasting, meaningful impact through innovation, sustainability, and a shared commitment to positive change.”
Kelly Henderson, Associate Director – Angus Connect
A key member of the Angus Connect leadership team, Kelly’s experience ranges from large-scale redevelopments to strategic planning, resulting in a proven capability to skillfully balance a diverse set of stakeholders from end users to C-suite, consultants and contractors. Kelly brings to her work a deep experience in facilities and technology planning, project management, government capital planning processes, and systems integration. She has been instrumental in expanding the Angus Connect practice into systems integration consulting, smart campus planning, and operational readiness. Kelly’s recent and current notable projects include Lakeridge Bowmanville Redevelopment ICAT Advisory, Toronto Western Hospital Surgical Tower, Smart Campus Planning for Saskatchewan Polytechnic and operational readiness consulting for the Dawson Creek & District Hospital Replacement Project.
Kelly:
“Having been a client, vendor, and now a leader at HH Angus, I’ve long respected the firm’s integrity, insight and commitment. I’m honoured to join such talented colleagues as we grow our business and deliver meaningful, lasting impact to the communities we serve.”
Nader Moslemian, Senior Manager | Senior Associate – Technology Division
Nader’s leadership in delivering mission critical facilities is founded on thirty-plus years of experience in the design, construction, commissioning, start-up, validation and qualification of mechanical systems. Deep engineering and technical knowledge, complemented by outstanding project management skills support Nader’s successful execution of projects. As a Senior Manager, he oversees a team responsible for the design of mechanical systems for critical spaces, including data centres, pharmaceutical, biotech, and laboratory buildings. Nader has also been instrumental in business development and the execution of laboratory projects; for example, a 20,000 ft2 Biosafety Level 2 wet laboratory fitout for Spec Lab. He is actively involved in mentoring junior members of his team and supporting their career development. A few of Nader’s recent and ongoing projects span a range of highly confidential facilities, such as a 4MW data centre in Alberta, a 27MW greenfield data centre in Ontario, and a 10MW data centre and a 140MW greenfield hyperscale data centre – both in Quebec.
Nader:
“Working at HH Angus during past decade, where I could contribute to the growth and development of the company’s mission critical facilities business, while helping young talents to blossom and gain huge experience in such a fast-paced industry, has been my real pleasure and provided me with a great satisfaction. I am grateful for this opportunity!”
Laura Sisson, Senior Manager | Associate – Health Division
Laura has consistently demonstrated strong leadership skills and a comprehensive understanding of design for healthcare spaces. Her deep knowledge of clinical requirements and hospital-specific codes supports a focus on effective design coordination and client satisfaction. As a Senior Manager, Laura oversees healthcare projects across the country and manages a team delivering multidisciplinary mechanical design from report/feasibility stage to the development of contract documents all the way through to contract administration. Her experience includes new builds, renovations and numerous P3 projects, in both pursuit and execution stages. Some of Laura’s notable projects include Royal Inland Hospital, Penticton Regional Hospital, Prince Edward County Memorial Hospital, Bayers Lake Community Outpatient Centre, and Centre hospitalier de l’Université de Montréal (CHUM).
Laura:
“Since my first day at HH Angus, I have been honoured to work on interesting and important projects with an amazing group of colleagues who foster a knowledgeable and collaborative work environment. I’m excited to move on to this next phase of leadership at the firm and continue to contribute to the success and positive future here at HH Angus.”
OUR NEW SENIOR ASSOCIATE
Will Evans, Senior Engineer – Health Division
Will’s leadership abilities have been amply demonstrated throughout the construction process, from conceptual design through to contract administration and project completion; for example, he provided on-site construction supervision as HH Angus' representative for Fort St. John’s greenfield hospital in northern British Columbia. Will’s high-level responsibilities include design and coordination of power, lighting and communications systems. He came to HH Angus with experience in commercial tenant projects, municipal infrastructure and controls automation, all of which served to broaden his skills and sector knowledge. Will’s notable projects include UHN’s Toronto Western Hospital New Surgical and Patient Tower, UHN’s Toronto General Hospital Rapid Assessment Centre, Centre hospitalier de l’Université de Montréal, Fort St. John Hospital and Peace Villa, and General Electric’s ‘Brilliant Factory’.
Will:
“I would like to thank HH Angus for the opportunity to work on such exciting projects and with such good people. I look forward to continuing my journey here.”
OUR NEW ASSOCIATES
Adrian Rybak, Senior Manager – Montreal/Ottawa Region
Adrian has been active in expanding HH Angus’ presence in Quebec while playing an important role in training and mentoring his team of engineers, designers, and project managers in HH Angus’ Montreal and Ottawa offices. His strong belief in the transformative power of digital technologies in the design and construction industry drives the widespread adoption of BIM across the firm, fostering interdisciplinary collaboration and improving project efficiency. Adrian challenges the Montreal/Ottawa team to use data, tools, and analysis before the design phase of a project to inform better decision-making, enabling clients and design teams to understand the project's context, goals, and constraints early on. Adrian’s notable projects include the Children’s Hospital of Eastern Ontario Integrated Treatment Centre, the Whitby Health Centre, various recreational and cultural facilities in Ontario, multiple Design/Build pursuits and, recently, supporting the new Smart Centers Head Office tower.
Adrian:
“An investment in knowledge pays the best interest — and at HH Angus, we're fortunate to have a strong culture of mentorship and knowledge sharing. I'm truly excited to be a part of it.”
Behnam Jowkar, Senior Manager – Health Division
Behnam has assumed increasingly senior leadership roles in HH Angus’ Health Division. His deep experience in HVAC, hydronic heating and cooling systems, fire protection, and control systems for healthcare facilities has made him a valuable member of multi-disciplinary project design teams. Behnam manages a team responsible for code-compliant engineering calculations, developing fire protection, heating and ventilation REVIT and AutoCAD drawings, and engineering reports. He brings to each project specialized experience in the design of HVAC systems, mechanical design analysis of mechanical/architectural design and shop drawings for LEED compliance, as well as ventilation and heat loss calculations under CSA and ASHRAE requirements. Key projects in Behnam’s portfolio include UHN’s Toronto Western Hospital New Surgical and Patient Tower, Trillium Health Partners’ New Peter Gilgan Mississauga Hospital, and Kingston General Hospital Mechanical Infrastructure Upgrades.
Behnam:
“I want to thank HH Angus for this great opportunity. I am looking forward to being able to contribute more to the success and growth of HH Angus. I have found my calling here and I’m extremely excited to experience the future of our industry with HH Angus.”
Greg Snow, Generation & BESS Lead – Energy Division
Greg leads electrical design teams and provides technical leadership on projects in the energy sector, specializing in power systems, electrical infrastructure, and energy storage systems. With a passion for sustainable energy solutions and a strong background in electrical engineering design, he has contributed to numerous BESS and decarbonization projects, resulting in deep technical knowledge. Greg is skilled in navigating the complexities of project delivery, stakeholder engagement and regulatory compliance. His leadership approach emphasizes collaboration, mentorship, and fostering a culture of continuous improvement, enabling teams to achieve both technical and operational excellence. Some of Greg’s notable projects include the TD Centre’s Long Term Energy Plan, Creative Energy’s Beatty Street District Energy and Low Carbon Steam Plant, Cadillac Fairview’s East Harbour Utility Infrastructure Planning, and GM’s Kapuskasing Cold Weather Testing Facility Power Upgrade.
Greg:
"After nearly 15 years with the firm, I’m grateful for the opportunities I’ve had to learn, grow, and lead at HH Angus. It’s been a rewarding journey, especially working alongside such exceptional colleagues. I'm excited for what lies ahead."
Ted Benstead, Manager – Health Division
Ted’s technical background and detailed knowledge of BIM incorporation and execution in construction projects deliver considerable benefits to our clients’ projects. Through extensive experience with Revit, Ted provides clients with precise design data and well-supported solutions. His experience in all facets of project design and delivery, including Prime Consulting, P3 and Alliance projects has contributed to developing a breadth of team leadership and technical skills. Ted manages a team of skilled professional designers and engineers, whose work includes conceptual design, preparation of specifications, electrical design and coordination, report writing, project coordination, design implementation and contract administration. Some of Ted’s notable projects include Burnaby Hospital Phase 2 and BC Cancer Centre, Thunder Bay Regional Health Sciences Centre Cardiovascular Surgery Expansion, Centre Hospitalier de l’Université de Montréal, Jerry Coughlan Health and Wellness Centre, and Michael Garron Hospital Patient Care Centre PDC.
Ted:
“It is incredibly fulfilling to watch HH Angus grow and know that it is made possible by the drive and expertise of everyone who contributes to our work across the country. The future is bright for HH Angus, and I am grateful for the opportunity to participate in exciting new projects, to mentor staff, and help expand our business.”
Vishal Bhana, Senior Manager – Angus Connect
With 15 years of experience, Vishal has taken on a lead role for major projects, developing market sectors and contributing to continuous improvement initiatives across the firm. As a senior manager in our Connect Division, Vishal is focused on technology design in the healthcare, mission critical, and commercial sectors. His strong client management skills and ability to mentor employees have been instrumental in fostering a collaborative and innovative work environment. Through his leadership, Vishal has driven the development of market sectors and contributed significantly to the firm's capabilities, delivering innovative solutions to complex challenges. Notable projects for Vishal include the Dawson Creek and District Hospital Project, the New Surrey Hospital and BC Cancer Centre, and the BC Mental Health and Substance Use Red Fish Healing Centre Expansion Business Plan.
Vishal:
“It’s incredibly fulfilling to collaborate with team members and clients on projects that serve our community and leave a legacy for many decades. I am honoured to be part of one of the leading consulting firms in the industry and am excited about our continued growth in British Columbia, as well as the opportunity to keep serving our clients with dedication and excellence.”